Abstract
Purpose
The purpose of this study is to investigate and analyse the potential of existing buildings in the UK to contribute to the net-zero emissions target. Specifically, it aims to address the significant emissions from building fabrics which pose a threat to achieving these targets if not properly addressed.
Design/methodology/approach
The study, based on a literature review and ten (10) case studies, explored five investigative approaches for evaluating building fabric: thermal imaging, in situ U-value testing, airtightness testing, energy assessment and condensation risk analysis. Cross-case analysis was used to evaluate both case studies using each approach. These methodologies were pivotal in assessing buildings’ existing condition and energy consumption and contributing to the UK’s net-zero ambitions.
Findings
Findings reveal that incorporating the earlier approaches into the building fabric showed great benefits. Significant temperature regulation issues were identified, energy consumption decreased by 15% after improvements, poor insulation and artistry quality affected the U-values of buildings. Implementing retrofits such as solar panels, air vents, insulation, heat recovery and air-sourced heat pumps significantly improved thermal performance while reducing energy consumption. Pulse technology proved effective in measuring airtightness, even in extremely airtight houses, and high airflow and moisture management were essential in preserving historic building fabric.
Originality/value
The research stresses the need to understand investigative approaches’ strengths, limitations and synergies for cost-effective energy performance strategies. It emphasizes the urgency of eliminating carbon dioxide (CO2) and greenhouse gas emissions to combat global warming and meet the 1.5° C threshold.
Keywords
Citation
Okonta, E.D. and Rahimian, F. (2024), "Examining approaches to investigating the United Kingdom’s existing building fabric in the pursuit of net zero targets", Urbanization, Sustainability and Society, Vol. 1 No. 1, pp. 78-114. https://doi.org/10.1108/USS-09-2023-0004
Publisher
:Emerald Publishing Limited
Copyright © 2024, Ebere Donatus Okonta and Farzad Rahimian.
License
Published in Asia Pacific Journal of Innovation and Entrepreneurship. Published by Emerald Publishing Limited. This article is published under the Creative Commons Attribution (CC BY 4.0) licence. Anyone may reproduce, distribute, translate and create derivative works of this article (for both commercial and non-commercial purposes), subject to full attribution to the original publication and authors. The full terms of this licence may be seen at http://creativecommons.org/licences/by/4.0/legalcode
1. Introduction
“Net Zero” means reducing greenhouse gas emissions to zero if possible or a point comparable to zero where remnants of emissions can be successfully absorbed by the natural environment without harm (United Nations, 2021). According to Reall (2021), the race to “Zero” aims for a vital, resilient and decarbonised planet, building momentum against future hazards for sustainable development. With a focus on balance, net zero emissions entail achieving balance and equilibrium between greenhouse gas (GHG) emissions released into the atmosphere and those expelled out of the atmosphere (Climate Council, 2021). The major greenhouse gases tied to the cause of global warming and climate change include carbon dioxide (CO2), methane (CH4), nitrous oxide (N2O), hydrofluorocarbons, perfluorocarbons and sulphur hexafluoride (SF6) (United Nations Environment Programme, 2022). The CO2 is the major contributor to climate change (IEA, 2021a). The global net-zero targets focus on reducing energy-related CO2 emissions to zero by 2050 and providing an opportunity to limit global temperature rise by 1.5°C (IEA, 2021b, p. 1). The UK (UK) has taken steps uniquely to pursue a net zero goal. This effort is reflected in the 10-point strategy for a green industrial revolution outlined by the HM government in 2020 (HM government, 2020). This strategy involves enhancing offshore wind capabilities, boosting the production of low-carbon hydrogen, expanding the utilization of nuclear energy, shifting towards emission-free transportation, improving eco-friendly public transit, striving for aviation and maritime emission neutrality, promoting sustainable infrastructure, deploying carbon capture and storage, conserving natural ecosystems and biodiversity and advancing environmentally responsible financial practices (HM government, 2020). GHG emissions among UK residents increased by 6% to over 505 million tonnes of CO2 equivalent between 2020 and 2021, and CO2 comprises 85% of GHG emissions in the UK, with methane (CH4) closely following greenhouse gas emissions (Office for National Statistics, 2022). Consumer expenditure stands as the largest individual contributor to emissions in the UK, constituting 27%, while electricity, gas, steam, air conditioning, manufacturing and transportation collectively account for 72% of emissions in 2021 (Office for National Statistics, 2022). The UK has thus far expanded its gross domestic product by 75% while reducing emission levels by 43%, becoming the first major economy in 2019 to adopt a legally binding commitment to achieve net zero greenhouse gas emissions by 2050 (Jones and Tam, 2023). In the UK built environment, including residential and commercial buildings, 77% are residential, 14% are commercial and 10% are public sector assets (Committee on Climate Change, 2018). The UK’s Environmental Audit Committee has highlighted that government policies have not provided sufficient momentum to address emissions reduction in this sector (UK Parliament, 2022). Space heating is one of the most polluting activities associated with the construction industry, accounting for 25% of total energy consumption in the UK (Committee on Climate Change, 2019). Further emissions from the building industry are caused by using grid electricity to power residences. In 2017, the UK construction industry accounted for 66% of total national power usage (Committee on Climate Change, 2018).
Most of the buildings that will be used in 2050 and 2070 to achieve the net zero target already exist (Dönmezçelik et al., 2023). In 2050, every existing building stock must be converted to almost zero-energy structures according to the European Union Energy Performance of Buildings Directive (European Union Law, 2018). Innovations that raise the affordability of integrating energy efficiency into newly constructed homes and enable retrofitting of existing properties to high energy efficiency standards will be necessary to meet these aims. This allows energy storage materials to be built into new construction properties and incorporated into modules/materials for retrofitting existing buildings. Supporting the integration of renewable energy-generating advances into new and existing properties (HM Government, 2018).
The study emphasizes the importance of investigating the existing building fabric; however, there is a research gap in the long-term performance monitoring of retrofitted buildings. A comprehensive understanding of the energy savings and carbon reductions achieved through different approaches is essential to validate retrofit interventions’ effectiveness and identify improvement areas (Crilly et al., 2012; Prabatha et al., 2020; Okonta, 2023). The study provides an overview of the approaches used to investigate the UK’s existing building fabric in the pursuit of net zero goals, including thermal imaging, in situ U-value testing, air tightness testing, energy assessment, condensation risk analysis and insulation assessment, and will seek to pursue these objectives:
To provide insights and recommendations for building owners, energy professionals and policymakers on effectively implementing these approaches to drive successful retrofit interventions;
To contribute to the body of knowledge on investigating the existing building fabric in the context of net zero targets, specifically focusing on the UK’s built environment; and
To support the UK’s efforts in achieving its net zero goals by providing evidence-based insights into effective approaches for transforming existing buildings’ thermal performance and energy efficiency.
This study uses a three-stage methodology to investigate the energy efficiency of the UK’s existing building fabric. First, a comprehensive literature review establishes the groundwork. Second, diverse case studies are selected to represent various investigative methodologies, covering thermal imaging, in situ U-value testing, airtightness testing, energy assessment and condensation risk analysis. Third, a case study analysis is conducted to provide real-world insights, and a cross-case analysis compares and contrasts two case studies within each investigative approach. This approach bridges theory with practical solutions for transforming the UK’s building fabric towards net-zero goals.
2. Retrofitting buildings
Retrofitting a building is a contemporary technique used to improve the reliability of a structure by improving its safety, durability and applicability with additional attributes and technology to the structure (Machhi, 2022). In much simpler terms, retrofitting a building entails modifying an existing structure to a new and stable one that could stand against or constitute a hazard to the environment. The growing energy consumption globally has created a need for more energy-saving strategies. With climate change constituting a significant hazard and a rising need for energy security, retrofitting buildings within the construction sector would reduce by 18.4% the contribution of these buildings to global GHG emissions (IPCC, 2014). Building retrofitting is also essential in reducing energy usage worldwide, as phase-change materials may deliver a boosted energy efficiency with minimal or no additional space required (Jelle and Kalnæs, 2017). Furthermore, building energy retrofitting provides adequate opportunities for reducing building energy consumption, improving cost and comfort, mitigating GHG emissions and aiding in the effective utilization of energy by buildings (Shaikh et al., 2017).
In Europe, historical buildings comprise 26% of the total population, with 14% constructed before 1919 and 12% between 1919 and 1945 (Tori, 2011). Over 40% of buildings in the UK were built before 1960 (Bogdan and Tudor, 2011), with 22.7% being residential buildings constructed before 1945 and 26.2% between 1945 and 1969 (EEA, 2020). These historic buildings have not undergone any form of energy retrofitting over the years. The average U-value of walls in residential buildings constructed between 1945 and 1969 is 1.39 W/m2K, while those built before 1945 have a U-value of 1.45 W/m2K (Hao et al., 2020). This has led to considerably higher energy consumption in these buildings compared to 21st-century buildings. Estimates suggest that improving thermal comfort could save 180 Mt of CO2 annually (Hao et al., 2020). Britain has over 28 million homes contributing to about 15% of the UK’s carbon emissions, and about ⅔ of the current building stock across has a huge possibility of standing by 2050; these buildings need to be retrofitted to meet the global net zero targets which have been set by the UK government (Sadler, 2022). Various researchers within the London School of Economics analysed that if all historically significant buildings within the UK were retrofitted, the energy consumption of England would see a drastic reduction of 1.3%, entailing a potential savings of about £1.7bn in energy consumption in seven years and decrease in CO2 emissions by 8.9 million metric tonnes (Saleem, 2020). Building retrofits could involve numerous processes, including installing better insulation, implementing energy-efficient glazing, upgrading lighting systems, optimizing water heating and integrating heat pumps (Project Drawdown, 2020). Improving the energy efficiency of buildings is both cost-effective and expeditious in reducing electricity demand, thereby decreasing carbon emissions and enhancing public health and environmental air quality in the local vicinity (Project Drawdown, 2020).
Moreover, incorporating techniques such as monitoring energy usage and water efficiency in real-time, implementing green roofing, enhancing indoor air quality (IAQ), optimizing operations and maintenance, enacting load curtailment initiatives, managing space utilization, using virtual building automation systems and ensuring public transparency and involvement are strategies that can be used during the renovation of a structure (Senseware, 2019). This approach transforms energy-efficient buildings into healthier, more productive environments (Senseware, 2019). Sadler (2022) opines that retrofitting aids in creating more thermally efficient buildings with high carbon efficiency and sustainability, making these buildings cheaper in the long haul and having minimal need for maintenance.
2.1 The building “fabric first” approach
Understanding the performance of the building fabric (also known as the building envelope) is critical for balancing energy consumption and plant size, maximizing comfort and minimizing condensation, overheating and thermal stress concerns. To reduce heat loss and enhance the energy efficiency of buildings, the building fabric is mostly considered the most important (Yang et al., 2022). The building fabric comprises all the components and materials that make up the building. This includes the walls, floors, roofs, windows and doors (Yang et al., 2022). The building fabric serves several functions, including protecting occupants from weather conditions like wind, rain, sun and snow. The building fabric is also essential for regulating the indoor environment regarding temperature, humidity and moisture content (Korjenic et al., 2011). Building fabrics contribute significantly to a building’s aesthetic and functionality (Klassen, 2006). Incorporating complexity and modern materials such as architectural fabrics, active shading and high-performance glazing can enhance structural performance while reducing energy consumption (Cole and Kernan, 1996). The building fabric usually contains openings to provide physical access for daylight admission and natural ventilation. This situation could hinder building performance in certain instances due to potential security risks, privacy concerns or noise disturbances associated with openings (Mohammad et al., 2022). Therefore, meticulous design considerations are essential, particularly regarding the junctions between elements comprising the building fabric, to prevent issues such as cold bridges between the internal and external environments (Wastiels and Wouters, 2012). Furthermore, the thermal performance of the building is influenced by the materials of the building fabric, which helps to conserve energy, improve the environmental friendliness of buildings, extend periods of indoor thermal comfort and reduce noise levels while fireproofing the building (Hung Anh and Pásztory, 2021).
2.2 Approaches for investigating building fabrics
Several researchers have proposed several methods for investigating building fabrics, including thermal imaging (Kylili et al., 2014; Lin et al., 2022), in situ U-value (Tejedor et al., 2019; O’Hegarty et al., 2021), air tightness testing (Carrié and Wouters, 2012; Bahnfleth et al., 1999), energy assessment (Cluett and Amann, 2015; Sun et al., 2021) and condensation risk analysis (Nath et al., 2022; Koo et al., 2018), which is critical to guarantee that the building improvement will not cause condensation and not impact IAQ.
2.2.1 Thermal imaging.
Thermal imaging observes and measures temperature fluctuations over surfaces (Lai et al., 2023). This method is valuable in assessing various facets of building conditions and behaviours, offering a visual insight into structural weaknesses. This, in turn, permits data acquisition and object analysis without necessitating sample removal or causing harm to the object’s integrity (Doshvarpassand et al., 2019). Thermal imaging cameras are primary tools for assessing various instruments, with multiple camera options available for thermal imaging applications. These cameras evaluate electricity distribution systems, electromechanical devices, buildings, roofs, tanks, pipes and valves (He et al., 2021). Conducting a thermographic survey can readily identify flaws in underground heating system pipes or tubes. When using a thermal imaging camera to identify inadequate insulation or energy leaks, the temperature contrast between the building’s interior and the outside should be at least 10°C (FLIR, 2011). However, a smaller temperature difference can suffice if a thermal imaging camera with high image resolution and thermal sensitivity is used. In colder climates, building inspections often occur in winter, while in warmer climates, where verifying proper insulation to retain cool air from HVAC systems indoors is crucial, the summer months offer an opportune timeframe for such thermal evaluations (FLIR, 2011).
Thermal imaging, or thermography, is crucial for visualizing heat energy through an infrared camera (Glaze and Save, 2018). The images captured by the camera show temperature variations across an area, from above absolute zero to net zero targets (Glaze and Save, 2018). These images display various colours or shades of thermal patterns based on the chosen colour palette (Glaze and Save, 2018). However, interpreting these patterns requires proper knowledge of the environmental conditions during image capture and the observed surface materials (Glaze and Save, 2018). The emissivity and reflectivity of an object are two key properties that affect the reliability of thermal imaging (Shuvo, 2022). Emissivity refers to an object’s ability to emit infrared radiation, while reflectivity is its ability to reflect it. Materials with high emissivity tend to have low reflectivity, and vice versa (Shuvo, 2022). Therefore, only materials with high emissivity can provide dependable thermal readings because materials with low emissivity can reflect the temperature of surrounding objects (Shuvo, 2022).
Older, non-retrofitted buildings often incur financial losses due to heat loss, particularly in cold spots, which can lead to dampness and condensation, potentially causing damage to building components (Roels and Tijskens, 2022). Thermal imaging is essential in identifying these cold spots and areas of heat loss caused by air leakage (Roels and Tijskens, 2022). This identification enables proactive measures to address the issue before it escalates (Roels and Tijskens, 2022). More so, thermal imaging is non-invasive and, as such, requires no dismantling or drilling of building elements. It is used without damaging the building fabric, even without considering the difficulty of locating draughts and air leakages. At the same time, moisture remains unseen unless it travels on walls, windows or roofs (Glaze and Save, 2018). Energy efficiency can also be improved by identifying unwanted heat transfer for later correction in lesser periods while providing documentation that could include inspection reports (Glaze and Save, 2018).
2.2.2 In situ U-value testing.
The In situ U-value testing explains how the thermal transmission characteristics of building materials like walls, floors and roofs are measured using a heat flow meter (Kim et al., 2022). The U-value measures how effectively building elements or fabric prevent heat loss to the external environment, influencing building energy efficiency (Zhang et al., 2020). Using the U-value in retrofit decision-making enables informed choices regarding the necessary insulation levels before retrofitting and evaluating the insulation’s performance after retrofitting (Zhang et al., 2020). The International Organization for Standardization recommends ISO 9869 as the in situ U-value determination standard (ISO) (Choi and Ko, 2019). The U-value can be established by several theoretical calculations, either using simplified methods such as defined by the BS EN ISO6946 or through numerical methods using detailed computer simulations which allow for a multifaceted heat flow software such as TRISCO or a combination of both methods (Kosmina, 2016).
Theoretically, one could establish the U-value by using a heat flow meter to gauge the heat movement across an element and noting the temperatures on either side under stable conditions (Kosmina, 2016). However, practically assessing the thermal resistance (R-value) or thermal transmittance (U-value) in contemporary residences presents challenges due to several factors (Choi and Ko, 2019). Building materials often lack uniformity, and their thermal conductivity remains uncertain. Accurately determining the dimensions of material layers frequently involves damaging methods, which might not always be viable (Choi and Ko, 2019).
The determination of the U-value involves several steps or elements. Firstly, it entails choosing the right heat flow meters with a minimum accuracy of ±5% (Zhang et al., 2020). It is important to note that larger heat flow meters offer greater sensitivity but are more challenging to mount on a wall. Secondly, selecting the appropriate temperature sensors is crucial, with a minimum accuracy of 0.3 K (Zhang et al., 2020). These sensors should be chosen based on the temperature to be measured; for instance, air temperature sensors are suitable if the U-value is specified as the ratio of the density of heat flow rate to the air temperature difference (Scarpa et al., 2017; Shinoda et al., 2021) rather than surface temperature sensors. Thirdly, calibration of the heat flow meter and temperature gauges is necessary, ensuring it has been conducted within the last 24 months. Fourthly, the measurement phase involves determining the measurement area, setting up temperature and heat movement sensors and collecting data. Finally, data analysis is performed (Zhang et al., 2020).
2.2.3 Air tightness testing.
Airtightness is the accepted technique for figuring out how much air is wasted overall due to leaks in the envelope or fabric of a building (Schreiber et al., 2021). Uncontrolled air leakage, accompanying ventilation energy losses, and occupant thermal comfort are all impacted by how airtight a building’s envelope is (Recart and Dossick, 2022). Gaining entry to an unoccupied dwelling is essential for an air-tightness examination. Air testers use specialized blower door testing equipment, essentially large fans affixed to an external opening, like a doorway (Zheng, 2020). The structure is subjected to pressurization through this setup while the testers assess pressure discrepancies.
Consequently, gaps and cracks permit external air pressure to infiltrate. Normally, the fan decreases the internal pressure of the dwelling to a minimum of −60Pa (Sadler, 2021). Using an anemometer, internal and external pressures are gauged alongside the fan’s effort to establish the pressure variation. Sadler (2021) states that this method aids in pinpointing air leaks, suggesting that the prevalence of such problems in sizable structures is likely to increase due to the extensive range of potential leakage points. New, large, non-domestic buildings (about 1,000 m2 floor space) are now obliged to fulfil a specific degree of airtightness under recent amendments to UK building regulations (Stamp et al., 2020). The traditional method of determining the airtightness of a building is through steady-state fan pressurization. According to some discussions in the airtight testing industry, the upper practical limit of large buildings that can be tested may be around 5,000 m2; this is because of technical issues with fan size, power requirements, transportation and noise (Feijó-Muñoz et al., 2019).
2.2.4 Energy assessment.
Energy assessment entails a thorough investigation into the energy usage of homes and the variables that influence it (Wang et al., 2022). It involves an inspection from the outside to the inside of a building, room by room (Bhamare et al., 2019). Tools like the power logger, ultrasonic flowmeter, T4 temperature data logger, airflow meter, thermal imaging cameras, IAQ meter, ultrasonic leak detector and flue gas oxygen omega data logger are all used for energy assessment within buildings (Enerteam, 2017). A room-by-room energy assessment is done throughout the house, along with a careful review of previous utility invoices. Many comprehensive energy evaluations commonly incorporate blower door examinations and a thermographic scan. Assessors might use tools such as blower doors, infrared cameras, furnace efficiency meters and surface thermometers to detect sources of energy wastage (Mauriello et al., 2019; Professional Home Energy Assessments, 2022). This process generally involves several steps: an initial interview, an outdoor survey, an indoor assessment, blower door and infrared assessments and a final evaluation of outcomes (Neagu and Teodoru, 2019). The final assessment outlines discoveries and specific measures for enhancing the energy efficiency of residences (Neagu and Teodoru, 2019). These measures aim to align homes with net zero objectives, targeting a 20% reduction in energy consumption and greenhouse gas emissions (Neagu and Teodoru, 2019). In a new energy assessment era in the UK, new rules on energy efficiency will be enacted in England and Wales. It would become mandatory that all commercially rented properties possess an energy performance certificate (EPC) of at least E for buildings’ energy consumption to be within acceptable limits (Handels Banken, 2018).
2.2.5 Condensation risk analysis.
Condensation is when airborne water vapour changes from a gaseous state to a liquid state. Within structures, this occurs when warm and damp air comes into contact with chilly surfaces that resist moisture (Sealy, 2022). Condensation can seriously diminish the insulation’s efficiency and harm a building’s structural integrity. An analysis of the risk of condensation on the roof or walls determines the possibility of interstitial condensation. Building control frequently requests these calculations to verify a person’s compliance with the regulations (BMI Group, 2021).
The change to solid walls can have issues that are not always obvious in achieving carbon neutrality and increasing building energy efficiency (Martel et al., 2021). Suppose nothing is in place to halt this vapour movement, either externally or internally with either a vapour control layer or render. In that case, the existing walls will remain breathable and capable of notwithstanding precipitation in their current state (Martel et al., 2021). When internal insulation is upgraded, the entire wall dynamic is altered because heat from the internal heat drive cannot dry the wall out, and vapour cannot travel through the wall without passing through the insulation and vapour control layer (Martel et al., 2021).
Surface condensation develops on exposed surfaces and contributes to surface stains and mould, which can typically be reduced with proper ventilation installation and use. Interstitial condensation can develop between layers of a structure, such as within roof, wall and floor components. This may result in the construction components degrading over time, decreasing the building’s long-term safety, increasing the likelihood of mould growth and lowering air quality (Ashby Energy Assessors, 2024). It can also potentially shorten the building’s lifespan; hence, interstitial condensation must be avoided, or adequate ventilation must be created to expel condensation (Libralato et al., 2019).
Numerous tools are accessible for conducting hygrothermal assessments, with the requirement that a reputable British industry organization like Building Research Establishment (BRE) in the UK endorse the software. The recommended applications are Flixo or Bisco for 2-D finite element analysis and Trisco for 3-D finite element analysis, which are used for analyzing surface condensation risk. These tools analyse the dew point line to ascertain the optimal placement of vapour control layers. They are crucial in cold bridging computation (Saied et al., 2022). For the assessment of interstitial condensation risk, tools like BuildDeskU (restricted to 1-D analysis using the Glaser method) and HU-FI Pro (providing dynamic simulation for vapour diffusion but confined to 1-D analysis focusing on straightforward scenarios rather than junctions) are used (Saied et al., 2022). These instruments are useful, but they still have limitations, as all of them, except the WUFI, identify vapour diffusion using the Glaser technique and assess the risk using steady temperatures. This technique fails to incorporate the potential impact of driving rain in its calculations, suggests unidirectional vapour movement (from inside to outside) and overlooks porosity or absorption measurements, consequently disregarding the potential risks related to moisture storage (Saied et al., 2022; Doda, 2020). Vapour diffusion determines how much water vapour can move through a building’s structure and can also pinpoint the optimal placement for effective vapour control and vapour-permeable membranes (Trifol et al., 2020). This ensures the well-being of the building envelope, whether it involves roofs or walls. This, in turn, enhances occupant health and diminishes factors such as heightened energy consumption in buildings, among other benefits (Trifol et al., 2020; Proctor Group, 2023). Table 1 summarizes the advantages and disadvantages of the approaches.
3. Methodology
This paper examines many investigative methodologies necessary for setting realistic and relevant objectives by assessing buildings’ present fabric performance and energy consumption. The research process followed a three-stage process presented in Figure 1.
3.1 Literature review
Firstly, when exploring methods to examine the UK’s existing building structures to achieve net-zero targets, this study drew upon a narrative literature review. A narrative literature review gathers existing research on a topic primarily through written text, summarizing and elucidating findings from various studies. This textual method essentially “tells the story” of the included studies, enabling an examination of similarities, differences and relationships within the data and an assessment of evidence strength (Popay et al., 2006). Although narrative synthesis lacks the structured methodology of other review types, it remains a valuable starting point for research, aiding in identifying trends and guiding future studies (Popay et al., 2006). This approach proves particularly beneficial when statistical or formal methods of data pooling are not feasible or suitable (Lisy and Porritt, 2016). A literature review is an objective and thorough examination and critical evaluation of available research and non-research literature related to the subject (Hart, 1998; Cronin et al., 2008). Its purpose is to provide readers with a comprehensive understanding of current literature on a particular topic and establish the groundwork for future endeavours, such as justifying further research. A proficient literature review gathers information about a specific subject from diverse sources and is composed unbiasedly, devoid of personal biases. It should encompass a well-defined search and selection methodology (Carnwell and Daly, 2001; Cronin et al., 2008). A well-structured review enhances logical flow and readability (Colling, 2003). The literature review was essential to explore the net zero targets, its benefits, retrofitting buildings, the building fabric, the approaches for examining the building fabric and their advantages and disadvantages. The narrative review was conducted using various academic databases, including but not limited to PubMed, Google Scholar and relevant journals. The study encountered limitations in conducting the desk review, a common aspect of such assessments. Challenges include the potential for bias and inaccuracies stemming from the quality of available data. Addressing contextual factors like local climate conditions and regulations may pose difficulties. Limited engagement with stakeholders could result in overlooking practical insights and incomplete evaluation of implementation challenges.
3.2 Case study selection
Using the case study method in this research allows for thoroughly examining complex real-world issues, especially when understanding phenomena deeply within their natural context. Case studies investigate specific phenomena using diverse data sources (Crowe et al., 2011). This method enables a comprehensive exploration of multiple dimensions, with implications for theory development and testing beyond the specific cases studied (Baxter and Jack, 2008). The case study method offers several advantages, including its ability to provide in-depth, detailed insights into complex phenomena or real-world situations (Yin, 1984). Case studies also allow for examining rare or unique cases, facilitating the exploration of topics that may be difficult to study using experimental or survey-based approaches (Crowe et al., 2011). However, case studies also have limitations. They often lack generalizability, as findings from a single case may not apply to other contexts or populations (Tellis, 1997). Case studies can be time-consuming and resource-intensive, requiring extensive data collection and analysis efforts (Yin, 1984). The case studies in Table 1 were chosen to reflect a variety of investigative methodologies used in investigating the existing building fabric. Each case study relates to a particular method, such as thermal imaging, in situ U-value testing, air tightness testing, energy evaluation and condensation risk analysis. The case study collection looks broad, embracing several geographical regions within the UK and various types of buildings, including residential, historic and institutional structures. This selection process enables a comprehensive grasp of investigative approaches in various building situations.
Furthermore, the study relied on ten (10) case studies, 2 (two) for each approach essential to understanding the current performance of the fabric and energy consumption of existing UK buildings, as summarized in Table 2. The study considered various criteria to determine which investigative approach best suits any case study. This included assessing whether the chosen approach could provide the necessary detail and depth to address the research questions effectively. Additionally, the study evaluated whether the investigative approach could be easily integrated with other building systems or infrastructure in the UK, thus minimizing building disruptions.
3.3 Case study analysis
The selection of case studies was based on previous research conducted by other scholars. These case studies were chosen because they provided valuable information about the various approaches for examining the building’s fabrics. By reviewing these case studies, the researchers aimed to understand the current performance of building fabric and energy consumption. The various assessment methods mentioned, such as thermal imaging, in situ U-value testing, airtightness testing, energy assessment and condensation risk analysis, were used to gather insights from the selected case studies. The case studies were evaluated using uniform steps such as background, problems, methods, findings and benefits. Thus, through the case study data analysis, the research strategy raises the research from abstract notions to real-world effects. The approach resonates with the context and challenges of net-zero building retrofits, aligning methodologically with the precision of the study, unearthing findings that tell a comprehensive story and ultimately offering practical solutions that can drive the transformation of the UK’s building fabric.
3.4 Cross case analysis
Furthermore, the study used cross-case analysis to compare and contrast the two case studies in each investigative approach. A cross-case analysis, as demonstrated in the studies of Shi Chi Hai Hutong in Beijing by Gu and Ryan (2008), pertains to comparing and contrasting different aspects of multiple case studies or situations. This analysis examines various buildings, locations or historical periods to identify common themes or patterns that connect them (Gu and Ryan, 2008). It allows for a comprehensive evaluation of the subject matter by considering differences and similarities across different cases or scenarios, contributing to a more holistic understanding of the topic. In the cross-case analysis, the study also considered the implications of both case studies in each investigative approach. Cross-case analysis is essential for understanding a subject from various angles. It helps find commonalities, differences and general trends, making research more reliable and insightful.
4. Results and discussions
This section analyses the approaches for understanding the building fabric and energy performance, including thermal imaging, in situ U-value testing, airtightness testing, insulation, energy assessment and condensation risk analysis.
4.1 Thermal imaging
4.1.1 Case study – The Grove House.
Background: The Grove House is on Roehampton Lane, Roehampton, London, SW15 5PJ. A low-rise structure with an 800 m2 ground floor and 320 m2 first floor that was initially constructed in 1777 by James Wyatt for Sir Joshua Vanneck was upgraded with heating and insulation in the 1990s to satisfy UK standards. It has two levels and 30 rooms. Building retrofits were carried out by Sustainable St Albans (Sustainable St Albans, 2020; Grove House, 2022).
Problems: Significant problems with temperature regulation and deteriorated mineral wood insulation were found across the structure. When the thermal imaging camera was deployed in 2020, it revealed regions that needed more attention, and numerous changes had been made in those areas.
Method: A thermal map of the entire structure was created using a thermal imaging camera to record the whole building and specific areas, like the ground and first-floor pipework and corridors. The temperature data were then translated from the thermal colour codes for use in building retrofits.
Findings: The most visible improvement was revealed in the underfloor heating, which was fixed by providing adequate pipework for uniform heat distribution within the building. The most noticeable difference is the underfloor heating, which is now functioning properly with the pipes giving even heat distribution, as seen in Figure 2.
Moreover, the mineral wood insulation, which is 100 mm thick, has degraded over the years and was removed from some locations during retrofitting to facilitate fireproofing, including metal lofts and access hatches. There was a distinct contrast between the ground-floor and first-floor corridors. The substitute loft insulation was not yet installed, and the photographs in Figures 3 and 4 justify boosting the insulation in this building area (which was not included in the restoration specification).
Benefits: Improved thermal performance of the building and reduced energy consumption within the building.
4.1.2 Case study – St. Julian Church, St. Albans, Hertfordshire, England.
Background: St. Julian’s Church, located in St. Albans, Hertfordshire, England, is a flint structure with stone accents believed to have been rebuilt in the 1950s to serve the cotton mill area. The church consists of a nave, a north aisle, a chancel and a partially offset tower. Notable features include 13th-century arches, a rib vault with moulded ribs supported by abaci columns and a distinctive singing desk. The interior showcases box pews, a two-deck pulpit, an organ in its gallery, a 14th-century chancel screen and the Lewknor family tomb adorned with ogee mouldings and carvings. The unique layout includes a separate priest’s reading station on the bottom deck of the pulpit (Historic England, 2009).
Problems: Increased energy consumption within the building was suspected to be a result of leakages in the roof and outdated boilers due to its period of installation in 1950.
Method: The thermal imaging camera is again used to encompass the entire structure and chosen spots for charting thermal bridges. A thermal bridge is a region where the building’s envelope offers reduced thermal resistance (Quinten and Feldheim, 2019), commonly due to construction limitations (Ge et al., 2021). Heat naturally seeks the path of least resistance, following from heated spaces to the exterior. This approach was used to identify regions with lower interior surface temperatures, potentially leading to issues like condensation, particularly at corners. It helps detect notably increased areas of heat loss and cold sections within buildings (Jiang et al., 2020). Figure 5 below shows a visible decline in heat loss when blinds are Figure 6 illustrates that while the church has efficient double glazing and a well-insulated roof, the metal window frames transmit cold air, and the main doors need a new bottom seal.
Findings: There was a substantial decrease in heat loss when window blinds were closed. Also, closed curtains and blinds could exhibit similar effects during double-glazing. Findings also revealed that the roof was properly insulated. Also, thermal imaging of the door shows that the seals need replacement as they allow seepage of cool air into the building. Also, although the windows were double-glazed, which is very effective, window frames conduct cold air, reducing the temperature and increasing energy consumption within certain locations in the building (Sustainable St Albans, 2019).
Benefits: These improvements are reflected in the cost of heating and energy consumption, which has drastically decreased by 15% from 16,200 kWh to about 13,500 kWh in three months.
4.1.3 Thermal imaging cross-case analysis.
Cross-analysis of the two thermal imaging case studies, “The Grove House” and “St Julian Church”, reveals several commonalities and differences in their approaches, findings and benefits:
4.1.3.1 Commonalities.
Both case studies use thermal imaging cameras as part of their methodology to evaluate the buildings’ thermal performance, identifying areas of heat loss and temperature variations. Their shared objective is to enhance the energy efficiency of the structures by addressing these thermal issues, guided by the thermal imaging results, which inform retrofitting decisions aimed at improving insulation and reducing energy consumption. Furthermore, thermal imaging identifies specific thermal deficiencies within both buildings, including problems related to insulation, heating systems and thermal bridges, all of which contribute to potential energy inefficiency. To effectively communicate their findings, both studies use visual documentation, including photographs, to illustrate the identified issues and the subsequent improvements made to the structures.
4.1.3.2 Differences.
Grove House (built in 1777) and St. Julian Church (rebuilt in the 1950s) differ in building type and age, influencing their thermal issues and retrofit processes. Grove House deals with underfloor heating and insulation issues, while St. Julian Church addresses heat loss through windows and thermal bridges tailored to their unique construction and use. The St. Julian Church study delves into thermal bridges related to window frames and blinds, while Grove House does not mention thermal bridge assessment. Grove House notes improved thermal performance without specific energy savings data regarding retrofit outcomes. At the same time, St. Julian Church achieves a significant 15% reduction in energy consumption over three months, dropping from 16,200 kWh to about 13,500 kWh.
4.1.3.3 Implications.
These case studies showcase thermal imaging’s adaptability in detecting thermal issues across various building types. They underscored the significance of addressing specific thermal problems for improved energy efficiency and reduced heating costs. Although both studies generally report positive retrofit outcomes, the extent of energy savings can differ significantly, emphasizing the importance of tailored solutions. The distinctions between these cases highlight the necessity of customized approaches to thermal retrofit projects based on each building’s unique characteristics.
4.2 In situ U-value testing
4.2.1 Case study – Lancaster West Estate, Central London.
Background: Building performance measurement was given by Build Test Solutions, a UK-based company, to help with building design, ensure construction quality and deliver important lessons and feedback to the Lancaster West Estate (Jack, 2021). Built in the middle of the 20th century, the Lancaster West Estate is a collection of medium-rise apartment buildings located in North Kensington in West London. It is in the Notting Dale suburb, which endured V-2 shelling during the Second World War. Due to the slum cleaning programme of the 1960s, it was built as municipal housing. The estate was created by architects Clifford Wearden and Peter Deakins in 1963–1964 as a significant Royal Borough of Kensington and Chelsea redevelopment project, but it was later significantly altered and scaled back in ambition East of Latimer Road tube station. (Glendinning and Muthesius, 1994; GmbH, Emporis, 2017).
Problems: The standards of insulation and artistry led to a negative impact on the U-value. Also, poorly fitted insulation, air leaks, cold badging from wall tiles and the diverse thermal properties of mortar joints all affect the U-value of the property. Government energy targets and affordable housing goals compelled authorities to re-evaluate the energy efficiency of their structures and search for alternative ways to add space to pre-existing structures. Due to this, the district heating system’s frequent breakdowns serving the finger blocks were visible (Jack, 2021).
Methods: The total thermal performance of the buildings was measured using SmartHTC to calibrate energy models that would be used in the retrofit design and identify homes where the performance is significantly different from what is expected. Measurements of pre-retrofit, thermal performance, air permeability, U-value, temperature and external thermography of buildings were measured. Also, post-retrofit, the same parameters were measured. After measurements were collected, retrofits would be made based on the findings (Jack, 2021). Figure 7 uses thermography to identify high heat loss areas and evaluates insulation levels with Heat3D and the U-Value Measurement System.
Results: improved thermal performance for dwellings and walls was better than pre-retrofitted walls, allowing for less costly specifications to achieve the net zero aims of the project. Also, U-value measurement results were added to the council asset management system (Jack, 2021).
Benefits: To make homes carbon-neutral by 2030, the energy required for heating and powering appliances was supplied by retrofitting the buildings with solar panels and air-sourced heat pumps. This, in turn, reduces energy consumption bills and levels.
4.2.2 Building research establishment examining various England residential bindings.
Background: As of 2021, in England, there were about 25 million homes (Statista, 2024). About two-thirds of these are cavity walls, while one-third are solid walls, such as those of solid brick, stone and concrete (Raushan et al., 2022). To effectively measure the energy consumption of the housing stock and to evaluate the potential for energy savings, it is critical to understand how walls of various sorts function in real-world settings. The Department of Energy and Climate hired the BRE to examine the thermal performance of walls in a variety of English residential buildings and to compare measured U-values to theoretically derived values (Hulme and Doran, 2014).
Problem: A lack of realistic estimates of building energy consumption has led to increased energy consumption and an inability to quantify the potential for energy efficiency improvements within these buildings.
Method: Heat fluxes (heat losses) through structural components like walls. U-values in about 300 solid walls, unfilled cavity walls and filled cavity wall houses were measured through the in situ monitoring of heat flow and temperatures. The field observations were also used to calculate theoretical U-values. The values that were calculated and measured were then contrasted. Homes previously visited as part of the 2010–2011 English Housing Survey (EHS) were chosen for these measurements (Hulme and Doran, 2014). Figure 8 shows the setup in an occupied home, with heat flux plates and temperature sensors supported by clamps and teleports and a tiny tag datalogger mounted on an exterior wall.
Results: The primary U-value examination in 2011–2012 unveiled a surprising finding regarding certain walls. These walls were initially categorized in the EHS as belonging to homes with predominantly uninsulated cavity walls, yet their U-values were unexpectedly low. This raised the possibility that these walls might have been insulated after all. There is a notable disparity between the average measured U-values (both mean and median) and the computed U-values for insulated hollow walls and the two types of solid walls. For insulated cavity walls, the measured U-values surpass the calculated values, implying that these walls seem to be performing less efficiently than the theoretical estimation suggests. Conversely, the measured U-values for solid walls are lower than the calculated values. Nevertheless, measurements indicate that uninsulated hollow walls correspond closely to the calculated values (Hulme and Doran, 2014). Figure 9 captures the heat distribution and performance of the equipment installed on a building wall, highlighting areas of thermal activity and potential heat loss or efficiency.
In this case, the wall’s thermal performance is exceptionally inconsistent; thus, choosing a representative measurement point at a temperature close to the average for the wall as a whole was required.
Benefits: One of the most significant factors influencing a home’s energy efficiency assessment is the amount of heat lost through the building’s envelope. Estimating and correctly diagnosing the amount of heat generated by structures is also crucial. The U-values of buildings are a factor that can overestimate a building’s energy efficiency because they represent a building’s original design values and thermal characteristics, which determine the thermal performance of an existing building. Therefore, the use of the above method aids in achieving net zero targets by accurately measuring a building’s energy consumption, hence assisting in the general reduction of energy consumed if the recommended retrofits are put in place (Park et al., 2021).
4.2.3 In situ U-value testing cross-case analysis.
Cross-analysis of the two case studies on in situ u-value testing, “Lancaster West Estate” and “Building Research Establishment (BRE) Examining Various England Residential Buildings” reveals commonalities and differences in their objectives, methods, findings and benefits:
4.2.3.1 Commonalities.
Regarding the in situ u-value testing, the two case studies, “Lancaster West Estate” and “BRE Examining Various England Residential Buildings”, share common objectives and methods. Both studies aim to assess thermal performance and measure U-values to enhance energy efficiency. They use in situ testing and various equipment for data collection, focusing on comparing measured U-values to theoretical values to inform energy-efficient retrofits. This emphasizes the significance of accurate U-value measurements for achieving energy-saving objectives.
4.2.3.2 Differences.
The two case studies, “Lancaster West Estate” and “BRE Examining Various England Residential Buildings”, differ in scope, building types, specific issues addressed and reported retrofit outcomes. “Lancaster West Estate” is limited to a particular housing estate in London, focusing on medium-rise apartment buildings and addressing issues like poorly fitted insulation and air leaks. It reports improved thermal performance and reduced energy consumption. In contrast, the “BRE Examining Various England Residential Buildings” study encompasses a broader range of residential buildings across England, including various building types and specific issues related to unexpected variations in U-values. However, it does not provide particular retrofit outcomes but emphasizes the importance of accurate U-value measurements for energy efficiency assessment.
4.2.3.3 Implications.
Both case studies emphasize the importance of accurate U-value measurements for assessing thermal performance and enabling energy-efficient retrofits. The “Lancaster West Estate” study showcases the practical application of U-value measurements in achieving energy-saving and carbon-neutral objectives. On the other hand, the “BRE Examining Various England Residential Buildings” study highlights the awareness of potential U-value variations and the necessity for meticulous monitoring and component analysis. While the challenges and solutions discussed are pertinent to various residential building types, the specific retrofit outcomes and goals may differ based on the project’s scope and objectives.
4.3 Air-tightness testing
4.3.1 Case study – Passivhaus Properties (108 dwellings tested).
Background: 108 dwellings in England were tested for potential air leaks by Build Test Solution. This work was completed in a reasonably short time as a reaction to the future home standard consultation, which suggests a lower limit of 1.5 m3/h/m2 @50Pa on the pulse method.
Problem: An issue that came up during previous lab-based tests of extremely airtight enclosures where agreement between the fan technique and pulse was being looked at. In each of these situations, we observe that the pressure pulse takes longer to reach its peak and that the decay rate also slows down significantly. Early implementations of the pulse technology experienced calculation difficulties for extremely airtight houses due to this shape change; timings became out of phase and the measurement procedure’s important “quasi-steady” portion was not reliably recorded (Zheng, 2020).
Method: All intended openings were sealed, doors and windows were closed and traps were filled following BS EN ISO 9972:2015’s Building Preparation Method. The connection between the blower door frame and the door frame was also taped up using airtight tapes during the blower door testing to reduce any leakage around the blower door unit. Once everything was in place, a certified test engineer ran a blower door fan test in pressurization and depressurization modes. Following the removal of the door fan, pulse tests were conducted soon after in each property, using the most recent hardware and software configurations (Zheng, 2020).
Findings: The data set shows an average difference between the blower door fan technique and pulse of −0.0003m3/h/m2 @4Pa. This amounts to an absolute percentage of 11%, broadly in line with expectation given the ISO 9972:2015 declared measurement uncertainty of the fan technique at 10%, the pulse measurement uncertainty at 5% and the additional uncertainty associated with Power Law extrapolation. This suggested areas for improvement in building envelopes due to uneven window and door seal sitting (Zheng, 2020). Figures 10, 11 and 12 reveal the air tightness testing in various locations by Build Test Solutions in England.
Benefits: Building envelopes must be properly sealed to enhance energy performance and distribution in diverse buildings, according to testing of a building’s airtightness. It is common knowledge that airtightness improves occupant comfort, IAQ, durability and liability for building proprietors. However, lowering energy use in the built world is a measurable objective (sadia_badhon, 2020). Also, setting a lower operational limit of 1.5m3/h/m2 @50Pa is not essential because Pulse can monitor extremely airtight houses just as well as the current blower door fan technology. Regardless of the measurement technique, measuring particularly airtight buildings might not be easy (Zheng, 2020).
4.3.2 Case study – ventilation assessment in cavity wall insulation in semi-detached bungalow.
Background: A semi-detached bungalow’s cavity wall insulation was installed by Dyson Energy Services using a preliminaries, assessment, design (PAS) 2035 compliant procedure. Therefore, having completed training offered by the Insulation Assurance Authority, Dyson did airtightness measurements before and after the retrofit, using Pulse to make an informed judgement on what further ventilation requirements were needed (Build Test Solutions, 2022).
Problem: Because cavity wall insulation can lessen a building’s air permeability, it is crucial to examine if enough ventilation will supply enough fresh air when the insulation is placed (Build Test Solutions, 2022).
Method: The air permeability of each room was measured using a pulse because, even though a building may have enough ventilation throughout, some rooms may not, putting them at risk of condensation or mould growth (Build Test Solutions, 2022). Figures 13 and 14 show air tightness testing with apparatus mounted with indoor readings.
Findings: The overall air permeability of the house before the retrofit was 1.69 ACH@4Pa (1/h). Following the refit, this figure significantly decreased to 1.15 ACH@4Pa (1/h), underscoring the importance of considering ventilation even with individual modifications. The measured air permeability of each room exceeded 1.5 ACH@4Pa (1/h), suggesting sufficient ventilation for the entire building and each specific room (Build Test Solutions, 2022).
Benefits: Measurements allowed for the insulation installer to adhere to PAS2035:2019 and to do the cavity wall insulation installation in a way that considers the dangers associated with a loss of ventilation. The measurements showed that the building and its rooms continued receiving enough fresh air through infiltration even after installing a single measure of cavity wall insulation. As a result, it was unnecessary to take extra ventilation measures like installing trickling vents and undercutting interior doors (Build Test Solutions, 2022).
4.3.3 Air tightness cross-case analysis.
The study performed a cross-analysis of the two case studies on air-tightness testing, “Passivhaus Properties” and “Ventilation Assessment in Cavity Wall Insulation in Semi-Detached Bungalow”, to identify commonalities and differences in their objectives, methods, findings and benefits:
4.3.3.1 Commonalities.
Both case studies share common objectives, methods and benefits of air-tightness assessment. Their primary aim is to evaluate the airtightness of buildings, identifying potential air leaks and ventilation needs. They use the pulse method for air-tightness measurement in both cases, conducting pre- and post-retrofit testing to assess the impact of insulation measures on airtightness. The studies underline the significance of measuring airtightness for enhancing energy performance, ensuring occupant comfort, maintaining IAQ and meeting building standards and regulations.
4.3.3.2 Differences.
The “Passivhaus Properties” and “Ventilation Assessment in Cavity Wall Insulation” case studies differ in scope and specific issues addressed. The former involves testing 108 dwellings in England to assess airtightness and compares pulse measurements to the fan technique, emphasizing technical challenges and measurement comparisons. In contrast, the latter focuses on a specific semi-detached bungalow to assess the impact of cavity wall insulation on air permeability, primarily highlighting the need for ventilation during insulation installation. The “Passivhaus Properties” study provides data on measurement differences. In contrast, the “Ventilation Assessment in Cavity Wall Insulation” case study focuses on pre- and post-retrofit air permeability values to showcase the effect of insulation on airtightness.
4.3.3.3 Implications.
Both case studies underscore the practical application of the pulse method for air-tightness measurements, offering a valuable tool for assessing and enhancing building performance. The “Passivhaus Properties” study addresses challenges in using Pulse for extremely airtight houses and confirms its effectiveness for monitoring such buildings. Conversely, the “Ventilation Assessment in Cavity Wall Insulation” case study highlights the need to prioritize ventilation during retrofits to safeguard IAQ and prevent condensation problems. Both studies emphasize the significance of measuring airtightness for achieving energy efficiency and meeting building standards and regulations.
4.4 Energy assessment
4.4.1 Case study – York Energy Demonstration Project.
Background: The York Energy Demonstration Project was used in Bell and Lowe’s (2000) study on energy-efficient housing modernization while discussing the implications for modernizing low-rise buildings in the UK. The assessed property was three- and two-bedroom, semi-detached, terraced houses built in the 1930s or 1950s. The structure consists of load-bearing cavity brickwork accompanied by a pitched tile roof. Ground floor constructions encompass a combination of solid and suspended timber elements. The floor area spans from 75 to 95 square meters. The loft insulation installed in the early 1970s varied in thickness, ranging from 25 to 100 mm, and no additional insulation was incorporated subsequently (Bell and Lowe, 2000).
Problems: The primary heating source for most houses was a gas fire in the main living area. At the same time, hot water was generated through an immersion heater connected to an insulated cylinder. A few homes, established in the 1970s, featured electric storage heaters and an electric fire in the main living room. These residences used an insulated storage cylinder to store hot water heated by an immersion heater designed to function during off-peak electricity periods (Bell and Lowe, 2000).
Method: Data from the building was collected for review, enabling the operator to perform various measurements that would allow him to define a “zero point” corresponding to the existing elements that still need to be improved. With the help of a thermal camera, the expert can measure the energy losses of the house. Adequate retrofits and renovation plans are made and then implemented and monitored before reports are made about the building’s energy consumption and improvements (Bell and Lowe, 2000). Table 3 compares gas consumption across all schemes, Table 4 details the heat loss coefficient (W/K) by element, and Figure 15 illustrates the energy characteristics of 30 house study groups.
Results: Retrofits of solar panels and air vents were done to the building to reduce energy consumption. It was discovered that the house that initially consumed 34,109kWh of energy before retrofit now consumed only 30,932 kWh after retrofit and had a consistent decline in energy consumption.
Benefits: The energy consumption of buildings can be reduced with proper retrofitting strategies for long-term care of buildings. From the base load of energy consumed, more power is consumed during winter and festive periods due to increased activities. More so, noon has minimal daily energy consumption. Hence, retrofits would aid in balancing energy consumption, while residents should be educated on energy-conservative techniques (Bell and Lowe, 2000).
4.4.2 Case study – Rose Cottage, Buttercrambe Estates.
Background: Energy performance certification was used to examine the energy efficiency of some buildings in England. The minimum energy efficiency standards working group, which included the National Trust (chair), Country Land and Business Association, Central Association for Agricultural Valuers, The Landmark Trust and other stakeholders, conducted this to provide evidence of the problems with EPC assessments for traditionally constructed buildings and identify the obstacles to effective energy efficiency improvements (Historic England, 2022). Figure 16 shows the Rose Cottage in 2021 (a three-bedroom semi-detached cottage from the 18th century built of coursed Hardwick stone with a brick lean-to and chimney stacks with a floor space of 78 m2.
Problem: Before the refurbishment, neither of the properties had been updated. The cottage had single glass throughout, no floor or wall insulation, open-hearth fires and rear boilers for heating. Their toilets and kitchens were old (Historic England, 2022).
Method: EPC baselines were completed to be compared to post-renovation evaluations. Rose Cottage G16 ratings (Historic England, 2022).
Results: Rose Cottage had a complete renovation with the same work criteria. This comprised loft insulation, replacement double-glazed windows, insulated doors, full interior dry lining with a Kingspan product, floor insulation and underfloor heating. Each home has a Mitsubishi Ecodan ASHP installed (Tables 5 and 6).
Benefits: Since then, greater benefits from solar gain through French doors have created a warmer environment. Newly advised measures include solar heating, 2.5 kWp solar photovoltaic panels and heat recovery systems for mixed showers (Historic England, 2022).
4.4.3 Energy assessment cross-case analysis.
The study performed a cross-analysis of the two case studies on energy assessment, “York Energy Demonstration Project” and “Rose Cottage, Buttercrambe Estates”, to identify commonalities and differences in their objectives, methods, findings and benefits:
4.4.3.1 Commonalities.
Both case studies share common objective of assessing and enhancing the energy efficiency of residential buildings in England. They use energy assessment methods to evaluate and compare the energy consumption of the buildings before and after retrofit activities, effectively measuring the impact of energy-saving measures. Both studies’ pre- and post-retrofit evaluations reveal that retrofits result in reduced energy consumption, improved energy performance and increased resident comfort, underscoring the benefits of such measures.
4.4.3.2 Differences.
The “York Energy Demonstration Project” and “Rose Cottage, Buttercrambe Estates” case studies differ in scope and energy sources. The former evaluates the energy-efficient modernization of houses from the 1930s and 1950s, focusing on heating systems, insulation and energy conservation. In contrast, the latter centres on renovating an 18th-century cottage, emphasizing air-source heat pumps and energy-saving technologies. The studies also differ in energy consumption data, with the York project providing detailed gas and electricity consumption figures before and after retrofit. At the same time, Rose Cottage uses EPC ratings to demonstrate energy improvements.
4.4.3.3 Implications.
Both case studies emphasize the benefits of energy-efficient retrofits in residential buildings, showcasing their positive impact on energy consumption and performance. The “York Energy Demonstration Project” stresses the importance of addressing heating systems, insulation and energy conservation to reduce energy consumption. In contrast, the “Rose Cottage” study highlights the potential for substantial energy performance improvements by installing modern technologies like air source heat pumps and solar panels. Both studies underscore the significance of conducting energy assessments and comparing pre- and post-retrofit evaluations to gauge the effectiveness of energy-saving measures.
4.5 Condensation risk analysis
4.5.1 Case study – Abbeyforegate Property, Shrewsbury.
Background: As part of the society for the protection of ancient Building Performance Survey, the property on Abbeyforegate underwent monitoring before and after renovations. The brick structure dates to circa 1820; it was originally mid-terrace, but one of the next buildings was removed. The structure has two floors and an attic dormer, with a usable floor space of roughly 60 m2. Shrewsbury’s “brick and a half thick” walls are 400 mm thick and constructed using a Flemish bond. The pointing is worn in many areas, and the lime-based mortar joints are around 10 mm wide (Browne, 2012).
Problem: Driven rain will collect in the open mortar joints if the wall is left as it is, so it needs to be repointed in a suitable material to shed water better. Traditional structures’ internal “common” bricks are frequently of lower quality, with less consistent shape and less frost resistance (Figure 17).
Method: This study examines interstitial moisture and the design U-value of the south-facing ground floor wall at the Abbeyforegate property in Shrewsbury using WUFI Pro 5.1 software. Thermal transmittance is modelled for pre- and post-renovation scenarios to determine whether critical moisture limitations are achieved at the internal wall insulation (IWI) and masonry wall contact. Investigations into microbial growth on the inside surface of the wall were not conducted (Browne, 2012).
Findings: These spaces would offer a capillary moisture break and lower the U-value of the wall. The wall is simulated as a solid wall with a complete link between all components because the voids will not be constant enough to form a continuous cavity. Worst-case situations for high U-values and moisture levels are likely to be shown in Figure 18, which is the pre-refurbishment assignment of material. After the renovation, the wall interior has an additional 40 mm of wood fibre insulation. This is divided into two layers, one 5 mm thick and the other 35 mm thick, allowing for monitoring the overall water content of the vulnerable inner 5 mm layer. As the wall gets thicker, the peak water content of the insulation contact falls. The mortar’s influence is demonstrated to have no impact on the 215 mm wall and only slightly raises the peak water contents of the 377 mm wall IWI.
Benefits: Reduced condensation risks within the structure were achieved, accompanied by suggestions to account for uncertainties in both internal and external climates. These include decreased indoor temperatures, alterations in ventilation levels, susceptibility to wind-driven rain and heightened rates of internal moisture generation. The condensation risk analysis also enhanced the property’s air exchange rate (Browne, 2012). Figure 19 illustrates the distribution of materials following refurbishment, specifically demonstrating how the simulation models wood fibre insulation.
4.5.2 Case study –retrofitting historic building in Paisley Town.
Background: The Townscape Heritage Conservation Area Regeneration Scheme of Renfrewshire Council is assisting with restoring a significant historic structure in the heart of Paisley that had become run-down. The building, a grade B-listed stone-faced terraced structure with a slated roof that dates to 1840, is located at 3 County Place and directly across from Paisley Gilmour Street train station (Proctor Group, 2023).
Problem: Condensation accumulated due to moisture infiltration via the roof and building walls. Two levels of the structure have been emptying for over 20 years, and upcoming roof, stone and window external repairs are needed to address the potential condensation concerns.
Method: To protect the roof from damage, a new roofing membrane, new leadwork and the reuse of the existing slates were all incorporated into the roof work. To maintain the aesthetic of the neighbouring buildings, all of the front elevation’s existing slates were kept, and CUPA H3 slate was added to the back. The roof’s sarking will be completely replaced (Proctor Group, 2023).
Results: In Figure 20, the new roofing structure allows for high levels of airflow in addition to the transportation of moisture vapour, making the formation of condensation in the roof space practically impossible, despite the complexity of the roof structures of historic buildings and the sensitive nature of the careful consideration of moisture management and condensation control. It has a very high level of air and vapour permeability; therefore, it can function in environments where airtight alternatives cannot (Proctor Group, 2023).
Benefits: Improved ventilation and thermal performance of the building while preserving and protecting historic building fabric Proctor Group, 2023).
4.5.3 Condensation analysis cross-case analysis.
The study performed a cross-analysis of the two case studies on condensation risk analysis, “Abbeyforegate Property, Shrewsbury” and “Retrofitting Historic Building in Paisley Town”, to identify commonalities and differences in their objectives, methods, findings and benefits:
4.5.3.1 Commonalities.
Both case studies aim to address condensation issues in historic or traditional buildings to enhance thermal performance and minimize condensation-related risks. They both deal with historic or traditional structures, acknowledging the delicate balance required for modernization while preserving heritage. Using energy modelling through simulations and software like WUFI Pro 5.1 and roofing membrane analysis, they assess U-values and moisture risk. The primary emphasis in both cases is on mitigating condensation risks, especially in areas susceptible to moisture infiltration.
4.5.3.2 Differences.
The “Abbeyforegate Property” and “Retrofitting Historic Building in Paisley Town” case studies differ in terms of location and building type, with one focusing on a brick structure in Shrewsbury and the other on a stone-faced terraced building in Paisley. The strategies to mitigate condensation also diverge, with the former emphasizing wall insulation and masonry properties and the latter addressing the roofing system with a focus on ventilation and moisture management. The “Abbeyforegate Property” concentrates on wall condensation risk, while the “Paisley Town” study deals with condensation in the roof space. Different materials and techniques are used, including wood fibre insulation and moisture behaviour analysis for the former, roofing membrane, CUPA H3 slate and new leadwork for the latter.
4.5.3.3 Implications.
Both case studies underscore the significance of managing condensation risk in historic or traditional buildings prone to moisture-related issues. Material selection, insulation and moisture management techniques are adaptable based on location and specific building components. Effective simulations and analytical tools are vital for assessing and mitigating condensation risk in heritage structures. Furthermore, improving the thermal performance of building elements, whether walls or roofs, is pivotal in reducing condensation risk and preserving historic fabric.
5. Conclusions and recommendations
In conclusion, the study examined approaches to investigating the UK`s existing building fabric to pursue net zero targets. A fact from the survey is that most of the buildings that will be used in 2050 and 2070 to achieve the net zero target already exist. A fact from the study is that most of the buildings that will be used in 2050 and 2070 to achieve the net zero target already exist. UK emissions are 77% attributable to residential heating, 14% to commercial buildings, and 10% to public sector assets. This will negatively impact the Net Zero targets if the trend is not addressed. The significance of the study underscores the importance of comprehending the advantages, drawbacks and cooperative potentials of investigative methodologies in developing economical energy performance strategies. It highlights the pressing necessity to eradicate carbon dioxide (CO2) and GHG emissions to address global warming effectively and adhere to the 1.5°C threshold.
The study attempted to evaluate five investigative approaches to examining the UK’s existing buildings towards achieving the net-zero targets. The approaches investigated include thermal imaging, in situ U-value testing, airtightness testing, energy assessment and condensation risk analysis. Thermal imaging is a valuable tool for identifying heat loss, cold spots and areas of trailing heat loss due to air leakage. It is a non-invasive method that provides visual evidence of energy inefficiencies, allowing for proactive responses to improve energy performance. In situ U-value testing is essential for measuring the thermal transmission characteristics of building materials. It helps determine the efficiency of insulation and informs retrofit decision-making. Airtightness testing helps identify air leakage points and assesses the overall energy efficiency of buildings. It plays a crucial role in reducing energy consumption and improving occupant comfort. Energy assessment provides a comprehensive evaluation of energy usage in buildings, enabling informed decisions on energy efficiency improvements. Condensation risk analysis helps prevent moisture-related issues, such as surface and interstitial condensation, which can affect building performance and occupant health. The study also examined the pros and cons of each investigative approach and examined, through case studies, their practical implication for the building fabric.
Secondly, the findings from the various building performance evaluation approaches have revealed several significant benefits. These include identifying and resolving building temperature regulation issues, which is crucial for maintaining comfortable and energy-efficient indoor environments. Moreover, implementing retrofits, such as solar panels, air vents, insulation, heat recovery systems and air-sourced heat pumps, has significantly improved thermal performance while reducing energy consumption, a key factor in energy efficiency. The findings also underscore the negative impact of poor insulation quality and artistry on the U-values of the buildings, emphasizing the importance of addressing insulation issues. Additionally, the effectiveness of pulse technology in measuring airtightness, even in extremely airtight houses, is highlighted, showing its value in assessing and improving energy performance by identifying and addressing air leakage issues.
Furthermore, the successful use of high airflow and moisture management techniques preserved the historic building fabric, demonstrating the balance between modernization and heritage preservation. These findings are useful to building developers and owners. Policymakers can incentivize and support these approaches through regulations, financial incentives and support programs to drive positive change in retrofit projects and achieve energy efficiency goals.
Furthermore, this study’s findings may have limited generalizability to diverse regions with varying climates and construction practices. The effectiveness of retrofit interventions may be context-dependent, considering building types and material differences. The study may not have observed or analysed the full and lasting effects of the retrofit interventions it investigated, as the study timeframe might have been relatively short. Additionally, the study assumes that implementing the investigated approaches requires technical knowledge and financial resources. However, this assumption may not be applicable universally, as some individuals or entities may lack the necessary expertise or financial means to carry out the proposed retrofit interventions. Additionally, the study might not account for emerging technologies or unforeseen factors influencing sustainable retrofit practices. While offering valuable insights, the study’s scope and applicability should be considered within these limitations, emphasizing the need for ongoing research and adaptability in the evolving field of energy-efficient retrofitting.
Also, continued research and innovation in investigating the existing building fabric are vital. Further studies should focus on refining and developing new approaches to improve the accuracy and efficiency of assessments. To validate retrofit interventions’ effectiveness and identify improvement areas, long-term performance monitoring of retrofitted buildings is also necessary. By advancing knowledge in this field, researchers can inspire innovation in sustainable retrofit practices and contribute to ongoing improvements in energy efficiency.
Figures
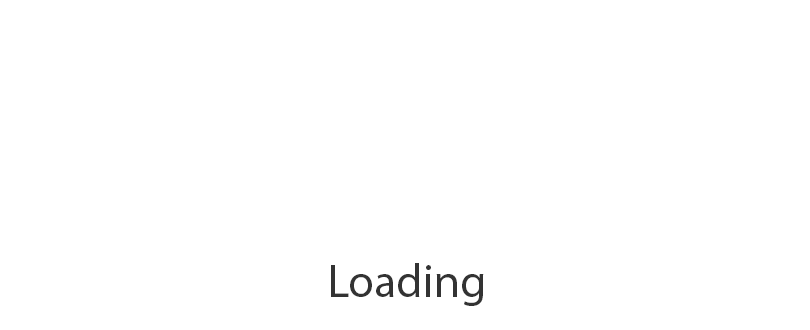
Figure 7.
Use of External thermography to highlight areas of particularly high heat loss (left) and Heat3D and the U-value Measurement System were used to measure U-values to understand the current condition of the walls, floors and ceilings and to provide information for the specification of extra insulation (right)
Analysis of the investigative approaches for examining the building fabric
Approaches | Advantages | Disadvantages |
---|---|---|
Thermal imaging | 1. Due to its non-contact safety, usefulness and effectiveness, it has many applications (Kylili et al., 2014) 2. It can be used as a communication tool to show home occupants where heat is lost from buildings (Boomsma et al., 2016) |
1. Images of certain items with variable temperatures are challenging to comprehend (Bednarkiewicz et al., 2021) 2. Unsuited for underwater objects and glass (Lin et al., 2022) |
In situ U-value testing | 1. High level of accuracy in testing building envelopes (O’Hegarty et al., 2021) 2. Ability to reveal the feasibility performance of buildings in various methods or time series of analysis (Tejedor et al., 2019) |
1. Although it stands as a non-invasive method, it can easily be categorized as part of tests that make use of heat flow meters (O’Hegarty et al., 2021) 2. Due to manual post-processing, it can be relatively slow (Tejedor et al., 2019) |
Air tightness testing | 1. Aids to improve overall operational and environmental quality, especially of high-raised buildings (Bahnfleth et al., 1999) 2. However, there is universal agreement that air tightness testing warrants attention in low-energy buildings as the energy impact of envelope airtightness varies on numerous aspects, including climate, building consumption, ventilation system type and air conditioning system usage (Carrié and Wouters, 2012) |
1. They stand to have some level of uncertainty in tall buildings concerning wind pressure and stack effect (Bahnfleth et al., 1999) 2. Parallel to this, worries about interior air quality and structural damage have sometimes led to additional regulations beyond the conventional ventilation criteria (Carrié and Wouters, 2012) |
Energy assessment | 1. It serves as an integral component of water utility performance evaluation (Bylka and Mroz, 2019) 2. Beyond energy savings, improved energy efficiency has several advantages, such as multi-family efficiency, health and comfort, as well as improved work efficiency (Cluett and Amann, 2015) |
1. Assessment could be poorly done, yielding poor and unreliable resources (Carley and Konisky, 2020). 2. Consistent changes in technology, tariffs and facilities affect recommendations that make previous audits outdated (Sun et al., 2021) |
Condensation risk analysis | 1. Precise evaluation of potential surface and interstitial condensation is crucial not only for mitigating the detrimental impact of moisture within building structures but also for ensuring a mould-free and healthful indoor environment (Mumovic et al., 2006) 2. To identify potential risks to building systems (components) in various climatic systems while potentially informing building regulations (Nath et al., 2022) |
1. It has many calculation methods, with two (Glaser annual calculation and monthly non-transient heat and moisture calculation methods) prone to many errors (Nath et al., 2022) 2. The accuracy of condensation risk evaluation is dependent on the exactness of calculated or measured temperatures (Koo et al., 2018) |
Source: Created by authors
Various investigative approaches and their case studies
Investigative approaches | Cases studies |
---|---|
Thermal imaging | • The Grove House, Roehampton, London (Sustainable St Albans, 2020; Grove House, 2022) • St Julian Church, St Albans, Hertfordshire England (Historic England, 2009) |
In situ U-value testing | • Lancaster West Estate, Central London (Jack, 2021) • Building Research Establishment (BRE) examining various England residential binding (Hulme and Doran, 2014) |
Air tightness testing | • Passivhaus properties (108 dwellings tested) (Zheng, 2020) • Ventilation assessment in cavity wall insulation in semi-detached bungalow (Build Test Solutions, 2022) |
Energy assessment | • York Energy Demonstration (Bell and Lowe, 2000) • Rose Cottage, Buttercrambe Estates (Historic England, 2022) |
Condensation risk analysis | • Abbeyforegate Property, Shrewsbury (Browne, 2012) • Retrofitting Historic Buildings in Paisley Town (Proctor Group, 2023) |
Source: Created by authors
Reveals EPC assessment summary for Rose Cottage
EPC date | October 2012 | June 2015 | January 2016 | April 2019 |
---|---|---|---|---|
Rating | F36 | E39 | F22 | E44 |
Flor Area | 90 m2 | 137 m2 | 104 m2 | 124 m2 |
Walls | Sandstone, solid brick, timber frame | Sandstone/limestone, solid brick | Granite/whinstone | Sandstone/limestone, timber frame |
Roof | Partial roof insulation | No roof insulation | No roof insulation | Partial roof insulation |
Windows | Single glazed | Single glazed | Full secondary glazing | Full secondary glazing |
Main heating | Oil | ASHP | ASHP | ASHP |
Main heating controls | Programmer, TVRs and bypass | Programmer, TVRs and bypass | Programmer and room thermostat | Programmer and at least two room thermostats |
Lighting | 86% low energy lighting | 89% low energy lighting | 38% low energy lighting | 83% low energy lighting |
Source: Historic England (2022)
Comparison of gas consumption – all schemes
Before improvement | Improvement to tenants choice standard | |||||
---|---|---|---|---|---|---|
Group | Gas | Elec. | Total | Gas | Elec. | Total |
kWh | kWh | kWh | kWh | kWh | kWh | |
Experimental | 26,946 | 7,944 | 34,890 | 27,864 | 3,246 | 31,110 |
Control | 26,290 | 7,818 | 34,109 | 27,851 | 3,081 | 30,932 |
Difference | 656 | 126 | 781 | 13 | 165 | 178 |
Source: Bell and Lowe (2000)
Heat loss coefficient (W/K) broken down by element
Element | Before | After | ||
---|---|---|---|---|
Mean | % | Mean | % | |
Floor | 25 | 11 | 25 | 17 |
Wall | 83 | 37 | 37 | 25 |
Windows and doors | 47 | 21 | 40 | 28 |
Roof | 20 | 9 | 9 | 6 |
Fabric heat loss coefficient | 125 | 78 | 111 | 77 |
Ventilation heat loss coefficient | 50 | 22 | 34 | 23 |
Total heat loss coefficient | 225 | 100 | 145 | 100 |
Source: Bell and Lowe (2000)
Reveals EPC assessment summary for Rose Cottage contd
Feature | Description | Rating |
---|---|---|
Wall | Sandstone or Limestone, as built, no insulation (assumed) | Very poor |
Wall | Timber frame, as built, partial insulation (assumed) | Average |
Roof | Pitched, 150 mm loft insulation | Good |
Roof | Pitched, no insulation (assumed) | Very poor |
Roof | Flat, no insulation (assumed) | Very poor |
Window | Full secondary glazing | Good |
Main heating | Air source heat pump, radiators, electric | Good |
Main heating control | Programmer and at least two room thermostats | Good |
Hot water | From main systems | Very poor |
Lighting | Low energy lighting in 83% of fixed outlets | Very good |
Floor | Solid, no insulation (assumed) | N/A |
Secondary heating | Room heaters, dual fuel (mineral and wood) | N/A |
Source: Historic England (2022)
References
Ashby Energy Assessors (2024), “Part C - condensation risk – building regulations”, available at: www.ashbyenergy.co.uk/condensation-risk-analysis (accessed 11 April 2024).
Bahnfleth, W.P., Yuill, G.K. and Lee, B.W. (1999), “Protocol for field testing of tall buildings to determine envelope air leakage rate”, American Society of Heating, Refrigerating and Air-Conditioning Engineers, Vol. 105 No. 2.
Baxter, P. and Jack, S. (2008), “Qualitative case study methodology: study design and implementation for novice researchers”, The Qualitative Report, Vol. 13, pp. 544-559.
Bednarkiewicz, A., Drabik, J., Trejgis, K., Jaque, D., Ximendes, E. and Marciniak, L. (2021), “Luminescence based temperature bio-imaging: status, challenges, and perspectives”, Applied Physics Reviews, Vol. 8 No. 1, p. 11317, doi: 10.1063/5.0030295.
Bell, M. and Lowe, R. (2000), “Energy efficient modernisation of housing: a UK case study”, Energy and Buildings, Vol. 32 No. 3, pp. 267-280, doi: 10.1016/s0378-7788(00)00053-0.
Bhamare, D.K., Rathod, M.K. and Banerjee, J. (2019), “Passive cooling techniques for building and their applicability in different climatic zones – the state of art”, Energy and Buildings, Vol. 198, pp. 467-490, doi: 10.1016/j.enbuild.2019.06.023.
BMI Group (2021), “Condensation risk analysis”, BMI, available at: www.bmigroup.com/uk/support/flat-roofing-services/condensation-risk-analysis/ (accessed 13 May 2023).
Bogdan, A. and Tudor, C. (2011), “A comparative analysis of the energy performance certificates schemes within the European union: implementing options and policy recommendations”, ECEEE 2011 Summer Study Conf. Energy Effic. first Found. a low-carbon Soc, pp. 595-604.
Boomsma, C., Goodhew, J., Goodhew, S. and Pahl, S. (2016), “Improving the visibility of energy use in home heating in England: thermal images and the role of visual tailoring”, Energy Research and Social Science, Vol. 14, pp. 111-121, doi: 10.1016/j.erss.2016.01.005.
Browne, D. (2012), “The SPAB research report 3. The SPAB hygrothermal modelling: Interim report”, available at: www.spab.org.uk/sites/default/files/documents/MainSociety/Advice/SPAB%20Hygrothermal%20Modelling%20Report%2031.pdf (accessed 23 May 2023).
Build Test Solutions (2022), “Ventilation assessment in cavity wall insulation”, available at: www.buildtestsolutions.com/case-studies/ventilation-assessment-in-cavity-wall-insulation (accessed 23 May 2023).
Bylka, J. and Mroz, T. (2019), “A review of energy assessment methodology for water supply systems”, Energies, Vol. 12 No. 23, p. 4599, doi: 10.3390/en12234599.
Carley, S. and Konisky, D.M. (2020), “The justice and equity implications of the clean energy transition”, Nature Energy, Vol. 5 No. 8, pp. 569-577, doi: 10.1038/s41560-020-0641-6.
Carnwell, R. and Daly, W. (2001), “Strategies for the construction of a critical review of the literature”, Nurse Education in Practice, Vol. 1 No. 2, pp. 57-63, doi: 10.1054/nepr.2001.0008.
Carrié, F. and Wouters, P. (2012), “Rationale and pros and cons of various approaches for setting building airtightness requirements”, 7th International BUILDAIR-Symposium, May 11-12, 2012, Stuttgart, Germany.
Choi, D.S. and Ko, M.J. (2019), “Analysis of convergence characteristics of average method regulated by ISO 9869-1 for evaluating in situ thermal resistance and thermal transmittance of opaque exterior walls”, Energies, Vol. 12 No. 10, p. 1989, doi: 10.3390/en12101989.
Climate Council (2021), “What does net zero emissions mean? | Explainer”, Climate Council, available at: www.climatecouncil.org.au/resources/what-does-net-zero-emissions-mean/ (accessed 20 May 2023).
Cluett, R. and Amann, J. (2015), “Multiple benefits of multifamily energy efficiency for cost-effectiveness screening (report A1502)”, American Council for An Energy-Efficient Economy, available at: https://c2e2.unepccc.org/wp-content/uploads/sites/3/2016/03/a1502.pdf (accessed 23 May 2023).
Cole, R.J. and Kernan, P.C. (1996), “Life-cycle energy use in office buildings”, Building and Environment, Vol. 31 No. 4, pp. 307-317, doi: 10.1016/0360-1323(96)00017-0.
Colling, J. (2003), “Demystifying the clinical nursing research process: the literature review”, Urologic Nursing, Vol. 23 No. 4, pp. 297-299.
Committee on Climate Change (2018), “Reducing UK emissions: 2018 progress report to parliament”, available at: www.theccc.org.uk/wp-content/uploads/2018/06/CCC-2018-Progress-Report-to-Parliament.pdf (accessed 20 June 2023).
Committee on Climate Change (2019), “UK housing: fit for the future?”, available at: www.theccc.org.uk/wp-content/uploads/2019/02/UK-housing-Fit-for-the-future-CCC-2019.pdf (accessed 20 June 2023).
Crilly, M., Lemon, M., Wright, A., Cook, M. and Shaw, D. (2012), “Retrofitting homes for energy efficiency: an integrated approach to innovation in the Low-Carbon overhaul of UK social housing”, Energy and Environment, Vol. 23 Nos 6/7, pp. 1027-1055, doi: 10.1260/0958-305X.23.6-7.1027.
Cronin, P., Ryan, F. and Coughlan, M. (2008), “Undertaking a literature review: a step-by-step approach”, British Journal of Nursing, Vol. 17 No. 1, pp. 38-43.
Crowe, S., Cresswell, K., Robertson, A., Huby, G., Avery, A. and Sheikh, A. (2011), “The case study approach”, BMC Medical Research Methodology, Vol. 11 No. 1, p. 100, doi: 10.1186/1471-2288-11-100.
Doda, A. (2020), “Facades and condensation risk analysis – introduction”, Patrick Ryan Associates, available at: www.patrickryanassociates.com/facades-and-condensation-risk-analysis-introduction/ (accessed 2 April 2023).
Dönmezçelik, O., Koçak, E. and Örkcü, H.H. (2023), “Towards net zero emissions target: energy modelling of the transport sector in Türkiye”, Energy, Vol. 279, p. 128064, doi: 10.1016/j.energy.2023.128064.
Doshvarpassand, S., Wu, C. and Wang, X. (2019), “An overview of corrosion defect characterization using active infrared thermography”, Infrared Physics and Technology, Vol. 96, pp. 366-389, doi: 10.1016/j.infrared.2018.12.006.
EEA (2020), “EC, 2020, ‘2050 long-term strategy’ – European environment agency”, available at: www.eea.europa.eu/policy-documents/ec-2020-2050-long-term-strategy#:∼:text=The%20EU%20aims%20to%20be%20climate-neutral%20by%202050 (accessed 20 May 2023).
Enerteam (2017), “Measuring equipment for energy audit, energy evaluation. Energy Conservation Research and Development Center”, available at: https://enerteam.org/measuring-equipment-for-energy-audit-energy-evaluation.html (accessed 10 May 2023).
European Union Law (2018), “Directive (EU) 2018/844 of the European parliament and of the council”, available at: https://eur-lex.europa.eu/eli/dir/2018/844/oj (accessed 20 May 2023).
Feijó-Muñoz, J., Pardal, C., Echarri, V., Fernández-Agüera, J., Assiego de Larriva, R., Montesdeoca Calderín, M., Poza-Casado, I., Padilla-Marcos, M.Á. and Meiss, A. (2019), “Energy impact of the air infiltration in residential buildings in the mediterranean area of Spain and the canary islands”, Energy and Buildings, Vol. 188-189, pp. 226-238, doi: 10.1016/j.enbuild.2019.02.023.
FLIR (2011), “An informative guide for the use of thermal imaging cameras for inspecting buildings, solar panels and windmills. Thermal imaging guidebook for building and renewable energy applications”, available at: www.flirmedia.com/MMC/THG/Brochures/T820325/T820325_EN.pdf (accessed 20 May 2023).
Ge, J., Xue, Y. and Fan, Y. (2021), “Methods for evaluating and improving thermal performance of wall-to-floor thermal bridges”, Energy and Buildings, Vol. 231, p. 110565, doi: 10.1016/j.enbuild.2020.110565.
Glaze and Save (2018), “Thermal imaging for retrofitting older buildings | laze and Save”, available at: www.glazeandsave.co.uk/thermal%20imaging-for-energy-efficiency-retrofitting-in-traditional-buildings (accessed 15 May 2023).
Glendinning, M. and Muthesius, S. (1994), Tower Block: modern Public Housing in England, Yale University Press, Scotland, Wales, and Northern Ireland., New Haven and London.
GmbH, Emporis (2017), “Grenfell Tower, London”, EMPORIS, available at: https://web.archive.org/web/20170617013347/https://www.emporis.com/buildings/135152/grenfell-tower-london-united-kingdom (accessed 15 May 2023).
Grove House (2022), “Wikipedia”, available at: https://en.wikipedia.org/wiki/Grove_House
Gu, H. and Ryan, C. (2008), “Place attachment, identity and community impacts of tourism – the case of a Beijing Hutong”, Tourism Management, Vol. 29 No. 4, pp. 637-647.
Handels Banken (2018), “Energy performance certificate (EPC) property rules are changing | Handelsbanken”, available at: www.handelsbanken.co.uk/en/about-us/sustainability/epc-property-rules-survey (accessed 5 May 2023).
Hao, L., Herrera-Avellanosa, D., Del Pero, C. and Troi, A. (2020), “What are the implications of climate change for retrofitted historic buildings? A literature review”, Sustainability, Vol. 12 No. 18, p. 7557, doi: 10.3390/su12187557.
Hart, C. (1998), Doing a Literature Review: Releasing the Social Science Research Imagination, Sage, London.
He, Y., Deng, B., Wang, H., Cheng, L., Zhou, K., Cai, S. and Ciampa, F. (2021), “Infrared machine vision and infrared thermography with deep learning: a review”, Infrared Physics and Technology, Vol. 116, p. 103754, doi: 10.1016/j.infrared.2021.103754.
Historic England (2009), “Church of St Julian, non-civil parish”, available at: https://historicengland.org.uk/listing/the-list/list-entry/1027871 (accessed 20 May 2023).
Historic England (2022), “Energy efficiency and historic buildings. Energy performance certificates (EPCs) case studies. Swindon. Historic England”, available at: https://historicengland.org.uk/images-books/publications/eehb-epcs-case-studies/heag0307-epcs-case-studies/ (accessed 25 June 2023).
HM Government (2018), “Clean growth strategy: executive summary”, available at: www.gov.uk/government/publications/clean-growth-strategy/clean-growth-strategy-executive-summary
HM Government (2020), “Achieving net zero (HC 1035). National audit office”, available at: www.nao.org.uk/wp-content/uploads/2020/12/Achieving-net-zero.pdf (accessed 20 May 2023).
Hulme, J. and Doran, S. (2014), “In-situ measurements of wall U-values in English housing (output number 290-102). Director, housing stock performance, BRE housing and energy”, available at: www.academia.edu/100850937/In_situ_measurements_of_wall_U_values_in_English_housing (accessed 23 May 2023).
Hung Anh, L.D. and Pásztory, Z. (2021), “An overview of factors influencing thermal conductivity of building insulation materials”, Journal of Building Engineering, Vol. 44, p. 102604, doi: 10.1016/j.jobe.2021.102604.
IEA (2021a), “CO2 emissions. Global energy review 2021 – Analysis – IEA”, available at: www.iea.org/reports/global-energy-review-2021/co2-emissions (accessed 20 May 2023).
IEA (2021b), “Net zero by 2050 – analysis”, IEA, available at: www.iea.org/reports/net-zero-by-2050 (accessed 20 May 2023).
IPCC (2014), “Climate change 2014: synthesis report. Contribution of working groups I, II and III to the fifth assessment report of the intergovernmental panel on climate change [core writing team”, in Pachauri, R.K. and Meyer, L.A. (Eds), IPCC, Geneva, Switzerland, p. 151.
Jack, R. (2021), “Lancaster west estate major retrofit assessment”, available at: www.buildtestsolutions.com/case-studies/lancaster-west-estate-major-retrofit-assessment (accessed 23 July 2023).
Jelle, B.P. and Kalnæs, S.E. (2017), “Phase change materials for application in energy-efficient buildings”, Cost-Effective Energy Efficient Building Retrofitting, pp. 57-118, doi: 10.1016/b978-0-08-101128-7.00003-4.
Jiang, X., Lin, A., Ma, H., Li, X. and Li, Y. (2020), “Minimizing the thermal bridge through the columns in a refrigeration room”, Applied Thermal Engineering, Vol. 165, p. 114565, doi: 10.1016/j.applthermaleng.2019.114565.
Jones, C.P. and Tam, S. (2023), “Low carbon and renewable energy economy (LCREE) survey QMI”, Office for National Statistics, available at: www.ons.gov.uk/economy/environmentalaccounts/methodologies/lowcarbonandrenewableenergyeconomylcreesurveyqmi (accessed 15 May 2023).
Kim, S., Seo, J., Jeong, H. and Kim, J. (2022), “In situ measurement of the heat loss coefficient of thermal bridges in a building envelope”, Energy and Buildings, Vol. 256, p. 111627, doi: 10.1016/j.enbuild.2021.111627.
Klassen, F. (2006), “From the bazaar to space architecture: fabrics reshape material and spatial qualities of built environments”, TEXTILE, Vol. 4 No. 3, pp. 256-269, doi: 10.2752/147597506778691512.
Koo, S., Park, S., Song, J.-H. and Song, S.-Y. (2018), “Effect of surface thermal resistance on the simulation accuracy of the condensation risk assessment for a high-performance window”, Energies, Vol. 11 No. 2, p. 382, doi: 10.3390/en11020382.
Korjenic, A., Petránek, V., Zach, J. and Hroudová, J. (2011), “Development and performance evaluation of natural thermal-insulation materials composed of renewable resources”, Energy and Buildings, Vol. 43 No. 9, pp. 2518-2523, doi: 10.1016/j.enbuild.2011.06.012.
Kosmina, L. (2016), “Guide to in-situ U-value measurement of walls in existing dwellings in-situ measurement of U-value”, available at: www.bre.co.uk/filelibrary/In-situ-measurement-of-thermal-resistance-and-thermal-transmittance-FINAL.pdf (accessed 25 May 2023).
Kylili, A., Fokaides, P.A., Christou, P. and Kalogirou, S.A. (2014), “Infrared thermography (IRT) applications for building diagnostics: a review”, Applied Energy, Vol. 134, pp. 531-549, doi: 10.1016/j.apenergy.2014.08.005.
Lai, Y., Albadi, A., Liu, X., Davies, M., Hobbs, M., Willmott, J. and Zhang, Y. (2023), “Investigation of forced flow orientations on the burning behaviours of wooden rods using a synchronised multi-imaging system”, Proceedings of the Combustion Institute, Vol. 39 No. 3, pp. 4105-4113, doi: 10.1016/j.proci.2022.07.057.
Libralato, M., Murano, G., De Angelis, A., Saro, O. and Corrado, V. (2019), “Generation of moisture reference years for interstitial condensation risk assessment: influence of the meteorological record length”, Building Simulation Conference Proceedings, doi: 10.26868/25222708.2019.210832
Lin, C., Hur, J., Chao, C.Y.H., Liu, G., Yao, S., Li, W. and Huang, B. (2022), “All-weather thermochromic windows for synchronous solar and thermal radiation regulation”, Science Advances, Vol. 8 No. 17, doi: 10.1126/sciadv.abn7359.
Lisy, K. and Porritt, K. (2016), “Narrative synthesis: considerations and challenges”, International Journal of Evidence-Based Healthcare, Vol. 14 No. 4, doi: 10.1097/01.XEB.0000511348.97198.8c.
Machhi, Y. (2022), “All about retrofitting | what is retrofitting | why need retrofitting | advantages and disadvantages of retrofitting of building. CivilJungle”, available at: https://civiljungle.com/retrofitting/ (accessed 20 May 2023).
Martel, T., Rirsch, E., Simmonds, W. and Walker, C. (2021), “The monitoring of wall moisture in a property retrofitted with internal wall insulation”, Case Studies in Construction Materials, Vol. 14, p. e00520, doi: 10.1016/j.cscm.2021.e00520.
Mauriello, M.L., McNally, B. and Froehlich, J.E. (2019), “Thermporal: an easy-to-deploy temporal thermographic sensor system to support residential energy audits”, Proceedings of the 2019 CHI Conference on Human Factors in Computing Systems, New York, NY, Paper 113, pp. 1-14, doi: 10.1145/3290605.3300343
Mohammad, F., Mohd, A., Mohamed, Rasidi, M.A.R., Sapian, N. and Dahlan, D. (2022), “Review of openings with shading devices at naturally ventilated buildings”, Architectural Engineering and Design Management, Vol. 19 No. 5, pp. 1-17, doi: 10.1080/17452007.2022.2095553.
Mumovic, D., Ridley, I., Oreszczyn, T. and Davies, M. (2006), “Condensation risk: comparison of steady-state and transient methods”, Building Services Engineering Research and Technology, Vol. 27 No. 3, pp. 219-233, doi: 10.1191/0143624406bse163oa.
Nath, S., Dewsbury, M., Künzel, H. and Watson, P. (2022), “Mould growth risks for a clay masonry veneer external wall system in a temperate climate”, Atmosphere, Vol. 13 No. 11, p. 1755, doi: 10.3390/atmos13111755.
Neagu, O. and Teodoru, M. (2019), “The relationship between economic complexity, energy consumption structure and greenhouse gas emission: heterogeneous panel evidence from the EU countries”, Sustainability, Vol. 11 No. 2, p. 497, doi: 10.3390/su11020497.
O’Hegarty, R., Kinnane, O., Lennon, D. and Colclough, S. (2021), “In-situ U-value monitoring of highly insulated building envelopes: review and experimental investigation”, Energy and Buildings, Vol. 252, p. 111447, doi: 10.1016/j.enbuild.2021.111447.
Office for National Statistics (2022), “Greenhouse gas emissions, UK: provisional estimates:2021”, available at: www.ons.gov.uk/economy/environmentalaccounts/bulletins/greenhousegasintensityprovisionalestimatesuk/2021#:∼:text=1 (accessed 10 June 2023).
Okonta, D.E. (2023), “Investigating the impact of building materials on energy efficiency and indoor cooling in Nigerian homes”, Heliyon, Vol. 9 No. 9, p. e20316, doi: 10.1016/j.heliyon.2023.e20316.
Park, S., Kim, S.H., Jeong, H., Do, S.L. and Kim, J. (2021), “In situ, evaluation of the U-value of a window using the infrared method”, Energies, Vol. 14 No. 7, p. 1904, doi: 10.3390/en14071904.
Popay, J., Roberts, H., Sowden, A., Petticrew, M., Arai, L., Rodgers, M. and Duffy, S. (2006), “Guidance on the conduct of narrative synthesis in systematic reviews”, A Product from the ESRC Methods Programme Version, Vol. 1 No. 1, pp. 1-92.
Prabatha, T., Hewage, K., Karunathilake, H. and Sadiq, R. (2020), “To retrofit or not? Making energy retrofit decisions through life cycle thinking for Canadian residences”, Energy and Buildings, Vol. 226, p. 110393, doi: 10.1016/j.enbuild.2020.110393.
Proctor Group (2023), “Managing moisture and condensation in buildings”, available at: www.proctorgroup.com/wufi (accessed April 2, 2023).
Professional Home Energy Assessments (2022), “USA department of energy”, available at: www.energy.gov/energysaver/professional-home-energy-assessments (accessed 20 May 2023).
Project Drawdown (2020), “Building retrofitting. Project drawdown”, available at: https://drawdown.org/solutions/building-retrofitting#:∼:text=Building%20retrofitting%20encompasses%20several%20solutions (accessed 20 May 2023).
Quinten, J. and Feldheim, V. (2019), “Mixed equivalent wall method for dynamic modelling of thermal bridges: application to 2-D details of building envelope”, Energy and Buildings, Vol. 183, pp. 697-712, doi: 10.1016/j.enbuild.2018.11.004.
Raushan, K., Ahern, C. and Norton, B. (2022), “Determining realistic U-values to substitute default U-values in EPC database to make more representative; a case-study in Ireland”, Energy and Buildings, Vol. 274, p. 112358, doi: 10.1016/j.enbuild.2022.112358.
Reall (2021), “Reall commits to the race to zero campaign”, Reall, available at: https://reall.net/blog/reall-commits-to-the-race-to-zero-campaign/?gclid (accessed 25 June 2023).
Recart, C. and Dossick, S.C. (2022), “Hygrothermal behavior of post-retrofit housing: a review of the impacts of the energy efficiency upgrade strategies”, Energy and Buildings, Vol. 262, p. 112001, doi: 10.1016/j.enbuild.2022.112001.
Roels, S. and Tijskens, A. (2022), “The impact of wooden studs on the moisture risk of timber frame constructions”, Journal of Building Physics, Vol. 46 No. 4, doi: 10.1177/17442591221140470.
Sadler, G. (2021), “Air tightness testing | the what, when and how”, available at: https://buildpass.co.uk/blog/air-tightness-testing-the-what-when-how-buildpass/ (accessed 2 April 2023).
Sadler, A. (2022), “7 Things you might not know about building retrofits”, available at: https://buildpass.co.uk/blog/7-things-you-might-not-know-about-building-retrofits/ (accessed 2 April 2023).
Saied, A.E., Maalouf, C., Bejat, T. and Wurtz, E. (2022), “Slab-on-grade thermal bridges: a thermal behavior and solution review”, Energy and Buildings, Vol. 257, p. 111770, doi: 10.1016/j.enbuild.2021.111770.
Saleem, M. (2020), “How and why retrofitting old buildings is both necessary and profitable - ClevAir blog”, available at: https://clevair.io/en/blog/retrofitting-old-buildings-necessary (accessed 20 May 2023).
Scarpa, M., Ruggeri, P., Peron, F., Celebrin, M. and De Bei, M. (2017), “New measurement procedure for U-value assessment via heat flow meter”, Energy Procedia, Vol. 113, pp. 174-181, doi: 10.1016/j.egypro.2017.04.050.
Schreiber, H., Jandaghian, Z. and Baskaran, B. (2021), “Energy performance of residential roofs in Canada – identification of missing links for future research opportunities”, Energy and Buildings, Vol. 251, p. 111382, doi: 10.1016/j.enbuild.2021.111382.
Sealy, E.A. (2022), “The treatment of wood (lumber)-2% copper naphthenate in kerosene a treatment for decay fungi, insects-termites, ants and beetles”, Scholars Journal of Engineering and Technology, Vol. 10 No. 8, pp. 185-205, doi: 10.36347/sjet.2022.v10i08.001.
Senseware (2019), “Top 10 retrofit methods for sustainable buildings”, available at: https://blog.attuneiot.com/top-10-retrofit-methods-for-sustainable-buildings (accessed 20 May 2023).
Shaikh, P.H., Shaikh, F., Sahito, A.A., Uqaili, M.A. and Umrani, Z. (2017), “An overview of the challenges for cost-effective and energy-efficient retrofits of the existing building stock”, Cost-Effective Energy Efficient Building Retrofitting, pp. 257-278, doi: 10.1016/b978-0-08-101128-7.00009-5.
Shinoda, J., Mylonas, A., Kazanci, O.B., Tanabe, S. and Olesen, B.W. (2021), “Differences in temperature measurement by commercial room temperature sensors: effects of room cooling system, loads, sensor type and position”, Energy and Buildings, Vol. 231, p. 110630, doi: 10.1016/j.enbuild.2020.110630.
Shuvo, M.M. (2022), “Multi-wavelength pyrometry for emissivity mapping and accurate surface temperature measurement in powder bed fusion systems – ProQuest”, available at: www.proquest.com/openview/0bd3dd65bd170afef64bde7a9ba8f731/1?pq-origsite=gscholar&cbl=44156 (accessed 20 May 2023).
Stamp, S., Burman, E., Shrubsole, C., Chatzidiakou, L., Mumovic, D. and Davies, M. (2020), “Long-term, continuous air quality monitoring in a cross-sectional study of three UK non-domestic buildings”, Building and Environment, Vol. 180, p. 107071, doi: 10.1016/j.buildenv.2020.107071.
Statista (2024), “Number of dwellings in England 2001-2020”, Statista, available at: www.statista.com/statistics/232302/number-of-dwellings-in-england/ (accessed 20 May 2023).
Sun, H., Edziah, B.K., Kporsu, A.K., Sarkodie, S.A. and Taghizadeh-Hesary, F. (2021), “Energy efficiency: the role of technological innovation and knowledge spillover”, Technological Forecasting and Social Change, Vol. 167, p. 120659, doi: 10.1016/j.techfore.2021.120659.
Sustainable St Albans (2019), “Thermal imaging camera case study – St Julian`s church”, available at: https://sustainablestalbans.org/2019/02/19/thermal-imaging-camera-case-study-st-julians-church/ (accessed 14 March 2023).
Sustainable St Albans (2020), “Thermal imaging camera case study – grove house”, available at: https://sustainablestalbans.org/2020/11/19/thermal-imaging-camera-case-study-grove-house/ (accessed 13 March 2023).
Tejedor, B., Casals, M., Macarulla, M. and Giretti, A. (2019), “U-value time series analyses: evaluating the feasibility of in-situ short-lasting IRT tests for heavy multi-leaf walls”, Building and Environment, Vol. 159, p. 106123, doi: 10.1016/j.buildenv.2019.05.001.
Tellis, W.M. (1997), “Introduction to case study”, The Qualitative Report, Vol. 3 No. 2, pp. 1-14, doi: 10.46743/2160-3715/1997.2024.
Tori, A. (2011), “Historic buildings and city centres – the potential impact of conservation compatible energy refurbishment on climate protection and living conditions”, International Conference Energy Management in Cultural Heritage, Dubrovnic.
Trifol, J., Plackett, D., Szabo, P., Daugaard, A.E. and Giacinti Baschetti, M. (2020), “Effect of crystallinity on water vapor sorption, diffusion, and permeation of PLA-Based nanocomposites”, ACS Omega, Vol. 5 No. 25, pp. 15362-15369, doi: 10.1021/acsomega.0c01468.
UK Parliament (2022), “Emissions must be reduced in the construction of buildings if the UK is to meet net zero, MPs warn”, available at: https://committees.parliament.uk/committee/62/environmental-audit-committee/news/171103/emissions-must-be-reduced-in-the-construction-of-buildings-if-the-uk-is-to-meet-net-zero-mps-warn/#:∼:text=Environmental%20Audit%20Committee-,Emissions%20must%20be%20reduced%20in%20the%20construction%20of%20buildings%20if,meet%20net%20zero%2C%20MPs%20warn&text=From%20residential%20to%20commercial%20buildings,the%20UK’s%20greenhouse%20gas%20emissions (accessed 23 July 2023).
United Nations (2021), “For a livable climate: Net-zero commitments must be backed by credible action”, United Nations, available at: www.un.org/en/climatechange/net-zero-coalition (accessed 4 May 2023).
United Nations Environment Programme (2022), “Emissions gap report 2022. UNEP - UN environment programme”, available at: www.unep.org/resources/emissions-gap-report-2022 (accessed 10 May 2023).
Wang, W., Xiao, W. and Bai, C. (2022), “Can renewable energy technology innovation alleviate energy poverty? Perspective from the marketization level”, Technology in Society, Vol. 68, p. 101933, doi: 10.1016/j.techsoc.2022.101933.
Wastiels, L. and Wouters, I. (2012), “Architects’ considerations while selecting materials”, Materials and Design, Vol. 34, pp. 584-593, doi: 10.1016/j.matdes.2011.05.011.
Yang, X., Hu, M., Zhang, C. and Steubing, B. (2022), “Urban mining potential to reduce primary material use and carbon emissions in the Dutch residential building sector”, Resources, Conservation and Recycling, Vol. 180, p. 106215, doi: 10.1016/j.resconrec.2022.106215.
Yin, R.K. (1984), Case Study Research: Design and Methods, Sage, Beverly Hills, Calif.
Zhang, J., Wu, W., Li, C., Yang, M., Zhang, Y., Jia, D., Hou, Y., Li, R., Cao, H. and Ali, H.M. (2020), “Convective heat transfer coefficient model under nanofluid minimum quantity lubrication coupled with cryogenic air grinding Ti–6Al–4V”, International Journal of Precision Engineering and Manufacturing-Green Technology, Vol. 8 No. 4, pp. 1113-1135, doi: 10.1007/s40684-020-00268-6.
Zheng, K. (2020), “Pulse tests in very air tight Passivhaus standard building (version 1.0). Build test solutions”, available at: https://cdn.sanity.io/files/m16cqsyp/production/be4fff6fdbccc02db81646e90ab5c2d157f153d0.pdf (accessed 30 May 2023).
Further reading
Grove House (2023), available at: www.accessable.co.uk/university-of-roehampton/access-guides/grove-house (accessed 20 May 2023).